Polymeric biomaterials for bone regeneration
Introduction
Combining biology and materials engineering, bone tissue engineering provides a potential approach for bone tissue repair and regeneration, especially for the critical defects that cannot self-repair (1). Compared to the autogenous and allogenous bone grafts, engineered bone tissue based on scaffolds loaded with autogenous cells may eventually eliminate the problems of supply scarcity, immunogenicity and potential pathogen transfer (2). As such, bone tissue engineering has witnessed rapid development in recent decades.
In general, bone tissue engineering requires artificial scaffolds with physicochemical, structural, and biological properties mimicking natural extracellular matrix (ECM), which provides a suitable environment for cell recruitment, proliferation, differentiation, and eventually bone regeneration. Facing the complex and sensitive biological systems, ideal scaffolds should not elicit immunological reactions and degrade in a controllable way with non-toxic products that can be excreted through metabolism. Biological agents are also needed to be incorporated to promote the formation of new bone tissues. Furthermore, the macro- and micro- structures (e.g., porosity) of the scaffolds should also be carefully designed to provide an optimized microenvironment for cell functions as well as to maintain the diffusion of nutrients and metabolites.
To date, diverse materials have been evaluated and utilized as scaffolds for bone regeneration, including metals, bioactive ceramics and glasses, natural and synthetic polymers and their composites. Among them, polymers and their composites are considered as the most promising candidates for their advantageous biocompatibility and biodegradability over most metals and ceramics (3). More importantly, polymers possess highly flexible design capacity, and their various properties can be easily tailored to meet specific requirements through manipulating their chemical compositions and structures. A wide range of natural polymers, including collagen, gelatin, chitosan, and synthetic polymers such as poly(lactic acid) (PLA), poly(glycolic acid) (PGA), polycaprolactone (PCL), have been applied for bone tissue regeneration, and they are usually composited with each other or other inorganic materials, e.g., calcium phosphates (CaPs), to optimize their osteogenic performance (3,4).
This review aims to provide a brief overview on the selection and design of polymeric biomaterials, fabrication techniques for achieving desirable macro- and micro-structural features of scaffolds, and bioactive modification methods for promoting bone regeneration.
Linear polymers for bone tissue engineering
Linear polymers refer to single-chain macromolecules without branches or intramolecular links. Table 1 summarizes the characteristics of most commonly used natural and synthetic polymers for bone tissue engineering.
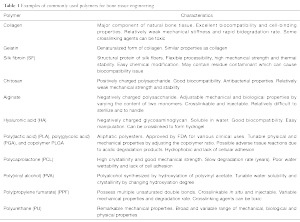
Full table
Natural polymers
Protein and polysaccharides are the major two naturally derived biodegradable polymers utilized in bone tissue engineering, with similarity with ECM to support cell adhesion and functions (2). As a major component of natural bone tissue, collagen, a fibrous protein, is an intuitive choice for bone tissue engineering. Among about 25 types of collagen, type I collagen is proven to offer the most suitable environment for osteogenesis (5). Pure collagen generally suffers from low mechanical strength and potential antigenic responses. Compositing with CaPs is a popular approach to enhance the mechanical strength of collagen. The composites show increased rigidity due to the strong interaction between the Ca-binding moieties of collagen and surface of CaPs (6). Collagen can be processed into diverse physical forms including hydrogels, sponges, and fibrous scaffolds. Wang et al. reported a thermoresponsive collagen/chitosan hydrogel gelated by an osteogenic medium substance β-glycerophosphate (7). Collagen in the composite was believed to improve cell spreading and proliferation, and chitosan to promote osteogenic differentiation of human bone marrow-derived stem cells (BMSCs). In another study, bone-mimicking nanofibrous collagen scaffolds were fabricated by electrospinning collagen with catecholamines and Ca2+ followed by mineralization with ammonium carbonate, which possessed Young’s modulus approaching that of cancellous bone (8). In vitro studies with human fetal osteoblasts demonstrated that the collagen composite scaffold improved cell proliferation and osteogenic differentiation than pristine collagen. Oriented collagen scaffolds prepared through a controlled freeze-drying process was also reported recently, which exhibited improved mechanical performance and better facilitated cell migration than random ones (9).
Gelatin is the denatured form of collagen. Despite the similarity with collagen, gelatin has higher water solubility and lower cost (2). Gelatin-based composites including gelatin methacryloyl (GelMA)/hydroxyapatite (HAp) hydrogel and gelatin/tricalcium phosphate (TCP) composite scaffolds have been reported to exhibit improved mechanical strength and excellent osteoconductive performance (10).
Silk fibroin (SF) is the structural protein of silk fibers with remarkable mechanical properties. Its degradation rate can be easily controlled by simply adjusting its molecular weight, crystallinity, and β-sheet structure. Many SF-based composites have been developed for bone tissue engineering. SF scaffolds incorporated with CaPs/silk powder or HAp showed improved mechanical properties and osteoconductivity (11). SF/HAp scaffolds were also combined with mesenchymal stem cells (MSCs) to enhance differentiation of MSCs and stimulate bone regeneration (12).
Chitosan is a positively charged polysaccharide with randomly distributed N-acetyl glucosamine and D-glucosamine. Therefore, chitosan can electrostatically interact with negatively charged molecules and membranes. Due to the weak mechanical strength, chitosan is often combined with inorganics like CaPs to fabricate stronger scaffolds with improved osteoconductive properties for bone tissue engineering (13). Small interfering RNA (siRNA) targeting bone-related proteins was also encapsulated in chitosan sponges to promote bone regeneration (14).
In contrast to chitosan, alginate is a negatively charged polysaccharide consisting of (1,4)-linked β-D-mannuronate and α-L-guluronate. Varying the content of two monomers can lead to different mechanical and biological properties of the alginate. Alginate is usually used as hydrogel by crosslinking with divalent cations (e.g., Ca2+) (15). As an example, an injectable alginate hydrogel system with mineralized collagen was reported recently for bone regeneration (16).
Hyaluronic acid (HA) is an anionic glycosaminoglycan with excellent viscoelasticity and water solubility. HA derivatives or HA-based composites have been widely used for bone tissue engineering. For example, photocrosslinked methacrylated HA hydrogel showed better mechanical properties, which was also loaded with simvastatin to promote osteogenesis (17).
Synthetic polymers
Compared to natural ones, synthetic polymers can be synthesized under more controllable conditions. Therefore, their physicochemical and biological properties, e.g., mechanical strength, degradation rate, and microstructure, are more predictable and reproducible, and desired properties can be conveniently obtained by wisely designing the segments and functional groups of the polymers.
Aliphatic polyesters, including PLA, PGA and their copolymer PLGA, are the most widely used polymers for bone tissue engineering. These polymers degrade in vivo by non-enzymatic hydrolysis and result in non-toxic degradation products. Their degradation rate can be readily tailored by altering the chemical composition, crystallinity, molecular weight, and distribution. However, these pure polymers have drawbacks such as insufficient cell recognition sites and osteoconductivity. To improve their performance, these polymers have been through PDA coating, grafting with bioactive molecules such as peptide and proteins, or compositing with HAp nanoparticles (3,18). For example, 3D-printed PLA scaffolds with PDA coating were reported to enhance the osteogenic differentiation of adipose-derived stem cells (ADSCs) (19). Electrospun PLGA scaffolds incorporated with gelatin/HAp were also reported to possess excellent biocompatibility and osteogenic activity (20). Compared to PLA, PGA and PLGA, PCL has higher crystallinity and hydrophobicity, and much lower degradation rate. Therefore, surface modification such as plasma treatment and loading of bioactive molecules is usually employed to overcome these disadvantages. PCL can also be copolymerized with other monomers to bear different functional groups, which can be further modified to enhance its interaction with cells for improved performance (21).
Poly(vinyl alcohol) (PVA) is a polyalcohol which is synthesized through hydroxylation of polyvinyl acetate. The degree of hydroxylation largely determines many characteristics of PVA, with higher degree of hydroxylation leading to lower water solubility and crystallinity (22).
Polyphosphoester (PPE) is a phosphate-containing polymer with potential osteoinductive capacity for bone regeneration. It was reported that the phosphoester groups of PPE promoted the osteogenic differentiation of human MSCs (23). Moreover, PPE was also used to modify the PLA surface to enhance the proliferation and functions of osteoblastic cells (24).
Polytrimethylene carbonate (PTMC) is an amorphous biocompatible polymer with low elasticity at room temperature. In a study which evaluated the performance of PTMC membrane in guided bone regeneration of rat mandibular defects, similar amount of newly generated bone was observed for PTMC, collagen, and PTFE membranes 2 weeks after implantation, predicting the potential use of PTMC in guided bone regeneration (25).
Biodegradable polyurethanes (PUs) are a series of synthetic polymers which gain increasing attention for bone tissue engineering. The properties of PUs can be tailored in a broad range by varying the chemical composition, segment ratio and structure, and molecular weight. PUs generally possess much better mechanical properties compared to other conventional biodegradable polymers (26). PUs are usually composited with inorganic materials and modified with bioactive substance to stimulate bone tissue regeneration (26).
Polypeptides used in bone regeneration include acidic poly(amino acids) such as γ-poly(glutamic acid) (γ-PGA) and poly(aspartic acid) and basic poly(amino acids) such as polylysine and polyarginine. As an example, a chitosan matrix was composited with γ-PGA to enhance its hydrophilicity and cytocompatibility (27).
Crosslinked polymers for bone regeneration
Hydrogels
Hydrogels are crosslinked network of hydrophilic polymers with micropores which trap a significant amount of water. With similar microstructure and composition as ECM, hydrogel materials are promising for bone tissue engineering, due to their advantageous mass transfer efficiency and capacity to encapsulate bioactive agents. The characteristics of hydrogels can be engineered by the chemical composition, crosslinking degree and swelling ratio. Increasing crosslinking degree generally leads to lower swelling ratio and stronger mechanical properties.
Natural polymers like alginate and HA can form hydrogel by chain entanglement, but chemical modification is usually applied to increase crosslinking degree (28). Alginate can be ionically crosslinked to form hydrogel in presence of multivalent ions (e.g., Ca2+) and used as a bone morphogenetic protein-2 (BMP-2) carrier to facilitate in vivo bone formation (29). In another study, HA-based hydrogels loaded with grow and differentiation factor-5 (GDF-5) facilitated sustained release of GDF-5 and significantly promoted osteogenesis in vivo (30). Synthetic polyethylene glycol (PEG) hydrogels are one of the most popular hydrogels for bone regeneration. Due to the antifouling properties of PEG, PEG-based hydrogels can resist non-specific adsorption, providing a clean background for biofunctionalization (23). Recently, hydrogels with multiple networks have attracted increasing attention due to their superior mechanical properties. The combination of strong covalent bonds and reversible physical bonds results in the formation of hydrogels with excellent ductility and toughness (15,31). Nonoyama et al. reported a double-network (DN) hydrogel composited with HAp nanocrystals, which showed high strength and toughness (31). The DN hydrogel exhibited remarkable osteointegration by forming a hydrogel/bone hybrid interfacial layer, and could be a promising material for bone regeneration.
Injectable and self-curing polymers
With the in situ crosslinkability, injectable and self-curing polymers can effectively alleviate many critical challenges associated with pre-fabricated bone implants (32). For example, injectable systems are less invasive and have shorter surgical time, which minimize tissue damage and may cause less scar formation. Injectable polymers can also be delivered into complex-shaped defects and then self-cure to form strong bonds with surrounding tissues. The curing process can be initiated by light, chemicals, and environmental stimuli (e.g., temperature, pH and ionic strength) (33). The curing rate can be optimized to ensure clinical efficacy while allowing sufficient time for premixing and injection and, in certain cases, encapsulation of bioactive molecules.
Bonzani et al. reported a two-component injectable PU system which could cure in situ by reaction between two pentaerythritol-based prepolymers (34). The injectable polymer displayed superior mechanical strength and supported the adhesion and proliferation of human osteoblasts, demonstrating great potential for bone regeneration. A thermoresponsive hydrogel was fabricated by composting triblock PEG/PCL/PEG copolymer with collagen and HAp (35). The composite hydrogel was injectable at room temperature, and formed gel at body temperature. The results proved the excellent biodegradability and bone regeneration performance of this injectable nanocomposite hydrogel. An injectable citric acid-based polymer, PEG maleate citrate could crosslink in situ to form elastomeric hydrogels which had excellent cytocompatibility and degradability and could be used as a delivery vehicle of cells (36). Poly(propylene fumarate) (PPF) is a unique polymeric material with multiple unsaturated double bonds available for crosslinking. PPF can be directly injected to the defect sites and then crosslinks in situ. When PPF scaffolds were loaded with CaPs and rhBMP-2, the synergistic effects of CaPs and rhBMP-2 promoted bone regeneration (37).
Naturally crosslinked polymers
Despite good biocompatibility, natural polymeric materials are often mechanically weak and unstable in the physiological environment during bone regeneration. For example, electrospun collagen fibrous scaffolds can rapidly disintegrate in aqueous solution (38). Crosslinking is a common approach to improve the stability and mechanical properties of natural polymer materials. Glutaraldehyde is a widely used chemical crosslinker which can react with the functional groups of both proteins and carbohydrates. Crosslinking with glutaraldehyde provided collagen sponges with better stability and cell attachment, yet impaired osteoblastic differentiation (39). While the cytotoxicity of glutaraldehyde is still controversial, other chemicals have also been used as crosslinkers (40). Genipin obtained from gardenia fruits is a natural crosslinker. Gelatin films crosslinked with genipin showed higher Young’ modulus and enhanced thermal stability, and their cytotoxicity was much lower than those crosslinked with glutaraldehyde (41). Genipin was also used to crosslink electrospun chitosan nanofiber membranes to satisfy the required degradation time frame for bone regeneration (42). Other natural agents such as proanthocyanidin were also reported to crosslink and stabilize collagen scaffolds (43).
Macro- and micro-structures of scaffolds for bone regeneration
Effect of structural and geometric cues on cells
Besides biochemical composition, the structure of scaffolds should also be wisely designed to provide suitable and stable support to cells during bone regeneration. It is generally accepted that adequate porosity with suitable pore size are essential for bone regeneration. Pore interconnectivity is also required to facilitate uniform cell seeding and transport of mass, nutrients and biological agents. However, high porosity may have negative impacts on the structural integrity and mechanical strength of scaffold, and may also accelerate its degradation during bone regeneration (44). Though challenging, an optimized balance among the structure, mechanical properties, and degradation rate is vital for the design of scaffolds.
The nano- and microscopic geometry and topography are also decisive factors affecting the spreading, shape, spatial arrangement and functions of cells (Figure 1) (45). For example, MSCs presented different spreading behaviors when cultured on substrates with adhesive islands of different size, among which the well-spread MSCs preferentially underwent osteogenic differentiation (46). Another study revealed that rectangular pattern with higher aspect ratio and pentagonal pattern with subcellular concave regions promoted osteogenic differentiation of MSCs (47). Polymer scaffold with microgrooves in radial arrangement was reported to facilitate guided osteoblast recruitment and enhance bone repair (48).
Polymer scaffolds with various geometries, such as porous scaffolds and fibrous matrices, have been prepared by using different processing techniques, including particulate-leaching, freeze drying, rapid prototyping (RP), phase separation and electrospinning (Figure 2). Scaffolds with hierarchical structures from micro- to macroscopic scale can be made by combining different techniques such as photolithography, microfluidics and micromolding (49).
Porous scaffolds
Incorporating porogen during fabrication is a conventional and convenient way to generate porous scaffolds, with porogen content and size determining the porosity and pore shape. Particulate leaching methods usually use water-soluble salts (e.g., sodium chloride, ammonium bicarbonate) as porogen, which can be leached out with water to create porous structures (26). This technique is usually combined with gas foaming, which uses gas as porogen, to achieve higher porosity and interconnectivity. A PLGA/HAp scaffold with 91% porosity was fabricated using gas foaming and particulate leaching technique (50). This porous scaffold showed superior pore interconnectivity and mechanical strength, and consequently higher exposure of HAp to transplanted cells at the pore surface, which effectively stimulated cell proliferation and osteogenic differentiation.
Freeze-drying is a dehydration process which creates porous structure by drying frozen ice crystals in polymer scaffolds. The porosity can be adjusted by manipulating the freezing rate and time, but the generated pores are relatively small compared with other processes. Using an emulsion foam freeze drying method, a porous PVA/CaP scaffold was prepared (51). The pore structure, mechanical strength, and biocompatibility were characterized to be qualified for bone tissue regeneration (51). Collagen/apatite composite scaffolds with tunable structure and mineral content were also fabricated by combining biomimetic self-assembly and controlled freeze drying process (52). The bone-forming capacity of such scaffolds predicts the potential of them for bone regeneration.
RP, also known as 3D printing, can directly “print” out objects with complex structure from computer generated data. The pore size, shape, and interconnectivity of scaffold can be precisely controlled and biological additives can be incorporated in the scaffolds during printing. However, fabricating structures of a few micrometers or submicron scale still remains challenging (53).
Micro/nanofibrous scaffolds
Collagen fibrils with diameters of 50–500 nm are the major ECM components of bone tissue. Therefore, fibrous scaffolds which structurally resemble the fibrous structure of ECM are preferred candidates for bone tissue engineering. Electrospinning is widely used for fabricating fibrous polymer scaffolds. The properties such as fiber size and stiffness can be controlled by the processing parameters including electric voltage and polymer concentration. A fibrous bilayer scaffold of PCL and gelatin/SG5 was fabricated using electrospinning, combining the advantageous mechanical property of PCL and higher mineralization performance of gelatin/SG5 for enhanced bone regeneration (54). Simultaneous electrospinning of collagen and electrospraying of HAp was also applied to prepare a fibrous collagen/HAp scaffold (55). The fibrous network allowed high cell infiltration and the presence of HAp improved the adhesion and proliferation of osteoblastic cells.
Thermally induced phase separation (TIPS) is a temperature-controlled phase separation process. The obtained structure consists of randomly oriented fibers, which is closer to the native 3D structure of bone ECMs than that fabricated by electrospinning. Using TIPS, a nanofibrous gelatin/biosilica scaffold was prepared, with characteristics mimicking native bone ECM (56). The hybrid scaffold showed good mechanical properties and biocompatibility.
Mechanosensing of cells on biomaterials
Cellular modulation by the elasticity of scaffolds
The elasticity represents to the resistance of a material to deformation and is a critical factor determining the behaviors of cells on a substrate. Cells generally have stronger adhesion on rigid substrates, while the adhesion is weaker on soft ones (57). This elasticity-dependent adhesion eventually leads to changes in cell morphology, migration, gene expression, and subsequent functions (58). For example, cells show more mobility with reduced spreading area on soft substrates compared to those on rigid substrates. On a substrate with gradient elasticity, the stronger contraction generated on more rigid region can direct movement of cells toward such region (59).
The substrate elasticity strongly regulates cell proliferation and apoptosis. For example, preosteoblastic cells cultured on stiff substrates showed higher proliferation and lower apoptosis rate compared to those on soft substrates (60). Pre-osteoblastic MC3T3-E1 cells on matrix with varying mechanical properties also showed better proliferation on stiffer matrix (61). More importantly, the differentiation of stem cells is also significantly affected by the elasticity of substrate. Stem cells preferentially differentiate into the cells of tissues with similar elasticity as the substrate. It has been reported that the osteogenic differentiation of MSCs was enhanced on substrates with higher Young’s modulus (62). Therefore, osteogenic differentiation of stem cells tends to improve on more rigid substrate. Indeed, it continues to improve with increasing elasticity within a certain range. However, it should be noted that the response of cells to substrate elasticity is non-linear and highly cell-dependent.
Polymer scaffold-mediated mechanical stimulation
Cells do not only passively adhere on the matrix, but actively interact with it by sensing the mechanical signals from the materials and dynamically remodeling their cytoskeletal networks. During bone regeneration, polymer scaffolds not only provide mechanical stability to maintain a stable microenvironment, but also provide mechanical stimuli to regulate the behaviors of attached bone cells through structural ligands, signaling peptides, proteinases, and inhibitors (63). When a cell attaches to a polymer scaffold, the contractile forces sensed by it result in tensile stress in the cytoskeleton, which further affects the behavior of cell. More rigid polymer leads to a stronger contractile response and vice versa. Polymers with a wide range of tunable mechanical properties have been studied as substrates for transducing forces to cells and promoting bone regeneration (64). Polyacrylamide (PA) hydrogels are most widely used for studying the effects of elasticity on cell behaviors, with their stiffness being readily adjusted by crosslinker content. Relatively rigid PA hydrogels mimicking collagenous bone promote osteogenic differentiation of stem cells (62). Poly(dimethylsiloxane) (PDMS) is also a popular elastomeric polymer with elastic modulus adjustable from tens of kPa to a few MPa. In a study, a PDMS substrate with stiffness gradient was created on by generating temperature gradients, which was used as an efficient scaffold to screen the appropriate stiffness for osteogenic differentiation of MSCs (65). The stiffness of PVA hydrogel can also be adjusted using a gradual freezing-thawing method (66). It was observed that BMSCs underwent neurogenesis and osteogenesis on the soft (~1 kPa) and stiff (~24 kPa) PVA hydrogels, respectively.
Bioactive modification of polymers for bone regeneration
Surface modification
The surface properties (e.g., surface chemistry and wettability) of polymer scaffolds significantly affect the adsorption of protein molecules and interactions between cell and matrix, which ultimately determine the cellular functions and quality of bone tissue formation. Engineering the surface properties is an effective way to improve the osteoconductive and osteoinductive performance of polymer scaffolds (Figure 3).
In general, most polymeric materials are hydrophobic and do not provide an ideal environment for protein adsorption and cell-matrix interactions. Various methods such as plasma treatment, corona discharge, and irradiation are therefore applied to generate functional groups such as hydroxyl and amine groups on the surface to improve their hydrophilicity (67). Grafting scaffold surface with different chemical functional groups can direct differentiation of human MSCs. For example, charged phosphate groups effectively promoted osteogenesis (23). This approach provides simple yet powerful technique to control the complex cell behaviors for bone tissue engineering.
Surface coating with inorganic materials is a popular way to modify polymers, especially synthetic ones, to enhance their biocompatibility and osteoconductivity. A tough DN hydrogel coated with HAps showed much improved osteointegration by forming a hybrid layer of hydrogel/bone at the interface (31). Polydopamine (PDA) coating is a novel universal coating technique. PDA-coated PLA scaffolds were proven to enhance cell adhesion, proliferation and promote osteogenesis (68). Furthermore, the catechol groups on the PDA coating can further react with primary amine and thiol groups to immobilize many other bioactive molecules (68).
Bioactive ligands such as peptides and polysaccharides can also be adsorbed or covalently grafted onto the surface to facilitate cell adhesion and spreading. Adhesion of cells on PLLA and PLGA scaffolds was greatly improved after grafting with an adhesive peptide RGD, and bone regeneration was promoted when combined with other bioactive molecules (69).
Bioactive substances delivery
Encapsulation of bioactive substances in polymer scaffolds and subsequent delivery in target region can provide important biological cues and signals to stimulate proliferation and migrate of target cells, and promote bone regeneration in vivo. The bioactive substances can be either physically encapsulated in or chemically bonded to the polymer matrix, to sustain a long-term and bioactive release. Various forms of delivery vehicles have been employed, including microspheres, hydrogels, porous and fibrous scaffolds.
BMPs are most commonly incorporated in polymer scaffolds to promote bone regeneration. Chitosan microspheres were used as delivery vehicle for BMP-2 in a porous HAp/collagen/PLA scaffold (70). The encapsulated BMP-2 was released in a temporally controlled process and effectively stimulated alkaline phosphatase activity of MSCs. In another study, an injectable and sustained release system of BMP-2 was designed using thermosensitive poly(phosphazene) nanoparticles and achieved 3-week sustained release of BMP-2, which promoted new bone formation (71). A polyelectrolyte film coating was also used as BMP-2 carrier, and the loaded amount of BMP-2 and its release rate could be controlled within a large range by adjusting crosslinking extent of the film and initial loaded concentration of BMP-2 (72). Heparin was also reported to be able to sustain the release of BMP-2 (73). When PLGA nanofibrous sheets were aminolysed and heparinized to be used as a delivery vehicle of BMP-2, sustained and active release of BMP-2 was demonstrated by the enhanced ALP activity and mineralization of MSCs, as well as the enhanced regeneration of bone tissue at defect area. Other growth factors such as fibroblast growth factor (FGF) are also loaded in polymer scaffolds to promote bone regeneration (74).
Antibacterial modification
Despite biocompatibility, many polymer scaffolds for bone engineering may suffer from implant failure due to bacterial infection. During implantation, bacteria can easily adhere to and colonize the polymer scaffold surfaces, ultimately leading to serious implant infection. Reducing bacterial adhesion to scaffold surface and bactericidal coating are two major methods to address the bacteria-associated concerns (75). Grafting with inert polymer brush, such as PEG, is a passive way to inhibit bacterial adhesion on the surface and prevent infection. However, it may also inhibit adhesion of mammalian cells (76). The reduced adhesion of osteogenic cells can be rescued by modifying the scaffold with RGD ligand peptide (77). In comparison, bactericidal coating is an active approach which can actually kill the bacteria and eliminate the root cause of infection. Silver is widely known to possess broad spectrum antimicrobial activity. A highly porous PLGA fibrous composite containing TCP and silver was fabricated using electrospinning (78). The encapsulated silver showed steady release and provided prolonged antibacterial effects. A cationic polymer, chitosan is a promising alternative to silver as an antibacterial agent. For example, chitosan and berberine were applied to coat a polyamide66/HAp scaffold (79). The combination of chitosan and drug berberine provided the scaffold with significant antibacterial efficacy. Hydrogels containing ZnCl2 and SrCl2 were also reported to effectively hamper the growth of bacteria on polymer scaffold for bone regeneration (80).
Immunomodulatory polymeric biomaterials for bone regeneration
As part of the foreign body responses, implantation of polymeric biomaterials often triggers a cascade of immune responses. This process can significantly impact the biological behaviors of bone cells and quality of the newly formed bone tissues, thereby limiting the effectiveness of many polymeric biomaterials. In general, an immune reaction that can provide an osteogenic microenvironment to promote osteogenesis is highly preferred for bone regeneration (81,82). Inadequate immune reactions can induce chronic inflammation at the implant site and result in the formation of a fibrous capsule around the implant, which isolates the implant from surrounding environment and inhibit adhesion of bone cells on the implant surface. Therefore, ideal polymeric materials for bone regeneration are expected to have the capacity to actively modulate local immune response to provide a suitable microenvironment for osteogenesis and osseointegration. This process involves inflammatory cells, primarily monocytes which subsequently differentiate into macrophages (monocyte-derived macrophages, MDMs) and play a critical role in determining whether bone regeneration and effective bone healing occurs (83).
The immune response to polymeric materials can be modulated by controlling the chemistry and surface characteristics as well as the microstructures of scaffolds. Material chemistry plays an important role in regulating cytokine release of monocytes by preventing the adsorption of serum protein and the downstream activation of complement and coagulation, so as to reduce inflammation (84). Similarly, surface features are critical in regulating macrophage polarization. For example, hydrophilic and anionic surfaces have been shown to inhibit long-term monocyte adhesion and to support anti-inflammatory responses of monocytes, while hydrophobic and cationic surfaces support foreign body giant cell (FBGC) formation and lead to local immune reactions (85). The topographical features also guide the response of immune cells to polymer materials. Recent studies have shown that topographical features promoted the adhesion and spreading of macrophages and favored their polarization toward M2 phenotype (86). The microstructural cues, including the pore size of porous scaffolds and fiber diameter of fibrous scaffolds, also play an important role in the immune responses (87). In addition, the mechanical property of materials such as stiffness has also been identified as a potential regulator of monocyte/macrophage activation (64,88). All of these material features should be finely tuned to meet specific requirements for the application for biomaterial design to alter the immune response.
Coupling with anti-inflammatory drugs is another method to achieve immunomodulatory effect of polymeric biomaterials. For example, dexamethasone loaded within polymer scaffolds can be released to reduce local inflammation induced by implants (89). Inorganic bioactive elements, depending on the composition and concentration, can also elicit a range of influences on the local microenvironment and immune responses. For example, Zinc and Strontium, known to stimulate osteogenesis, also regulate immune response in a concentration-dependent manner (90).
Concluding remarks
Dramatic progress has been made in recent decades in the application of polymeric biomaterials, both natural and synthetic, as scaffolds for bone regeneration. Among them, natural polymers are generally biocompatible and possess intrinsic biological cues to support cell adhesion, proliferation, and differentiation, but with weak mechanical strength and relatively fast degradation rate. Synthetic polymers, on the other hand, have more controllable physicochemical and biological properties, yet lack sufficient biological recognition signals. Therefore, these two categories of polymers are usually composited with each other or with inorganic materials to obtain an optimization of a diversity of properties, including biocompatibility, biodegradability, surface chemistry, geometric structure and mechanical property, to meet the specific requirements for bone tissue engineering. These polymeric materials can also be encapsulated with bioactive substance to realize controlled delivery of them to promote bone regeneration.
Although remarkable advances have been made, the ideal scaffolds for bone tissue engineering still remain to be developed. The biophysical and biochemical properties of polymeric materials should be further exploited to guide material design and fabrication. On the other hand, more experimental and theoretical insights into the mechanisms of geometry or mechanical sensing of the cells on the polymer scaffolds are needed, which will facilitate controllable manipulation of cell functions. Moreover, more comprehensive evaluations of these polymeric materials both in vitro and in vivo are still required to explore their potential as bone implants.
Acknowledgments
Funding: The authors are grateful to the funding support from the National Natural Science Foundation of China (81471790, 31530024, 81672213), Ministry of Science and Technology National Key Innovation Project (2016YFC1100203), Jiangsu Provincial Special Program of Medical Science (BL2012004), and the Priority Academic Program Development (PAPD) of Jiangsu Higher Education Institutions.
Footnote
Conflicts of Interest: All authors have completed the ICMJE uniform disclosure form (available at http://dx.doi.org/10.21037/aoj.2016.11.02). The authors have no conflicts of interest to declare.
Ethical Statement: The authors are accountable for all aspects of the work in ensuring that questions related to the accuracy or integrity of any part of the work are appropriately investigated and resolved.
Open Access Statement: This is an Open Access article distributed in accordance with the Creative Commons Attribution-NonCommercial-NoDerivs 4.0 International License (CC BY-NC-ND 4.0), which permits the non-commercial replication and distribution of the article with the strict proviso that no changes or edits are made and the original work is properly cited (including links to both the formal publication through the relevant DOI and the license). See: https://creativecommons.org/licenses/by-nc-nd/4.0/.
References
- Lanza R, Langer R, Vacanti J. Principles of Tissue Engineering, 3rd Edition. Academic Press, 2007.
- Pina S, Oliveira JM, Reis RL. Natural-based nanocomposites for bone tissue engineering and regenerative medicine: a review. Adv Mater 2015;27:1143-69. [Crossref] [PubMed]
- Puppi D, Chiellini F, Piras AM, et al. Polymeric materials for bone and cartilage repair. Prog Polym Sci 2010;35:403-40. [Crossref]
- Ma X, He Z, Han F, et al. Preparation of collagen/hydroxyapatite/alendronate hybrid hydrogels as potential scaffolds for bone regeneration. Colloids Surf B Biointerfaces 2016;143:81-7. [Crossref] [PubMed]
- Mizuno M, Shindo M, Kobayashi D, et al. Osteogenesis by bone marrow stromal cells maintained on type I collagen matrix gels in vivo. Bone 1997;20:101-7. [Crossref] [PubMed]
- Zhang Y, Reddy VJ, Wong SY, et al. Enhanced biomineralization in osteoblasts on a novel electrospun biocomposite nanofibrous substrate of hydroxyapatite/collagen/chitosan. Tissue Eng Part A 2010;16:1949-60. [Crossref] [PubMed]
- Wang L, Stegemann JP. Thermogelling chitosan and collagen composite hydrogels initiated with beta-glycerophosphate for bone tissue engineering. Biomaterials 2010;31:3976-85. [Crossref] [PubMed]
- Dhand C, Ong ST, Dwivedi N, et al. Bio-inspired in situ crosslinking and mineralization of electrospun collagen scaffolds for bone tissue engineering. Biomaterials 2016;104:323-38. [Crossref] [PubMed]
- Chen P, Tao J, Zhu S, et al. Radially oriented collagen scaffold with SDF-1 promotes osteochondral repair by facilitating cell homing. Biomaterials 2015;39:114-23. [Crossref] [PubMed]
- Visser J, Gawlitta D, Benders KE, et al. Endochondral bone formation in gelatin methacrylamide hydrogel with embedded cartilage-derived matrix particles. Biomaterials 2015;37:174-82. [Crossref] [PubMed]
- Yan LP, Silva-Correia J, Oliveira MB, et al. Bilayered silk/silk-nanoCaP scaffolds for osteochondral tissue engineering: In vitro and in vivo assessment of biological performance. Acta Biomater 2015;12:227-41. [Crossref] [PubMed]
- Liu H, Xu GW, Wang YF, et al. Composite scaffolds of nano-hydroxyapatite and silk fibroin enhance mesenchymal stem cell-based bone regeneration via the interleukin 1 alpha autocrine/paracrine signaling loop. Biomaterials 2015;49:103-12. [Crossref] [PubMed]
- Dhivya S, Saravanan S, Sastry TP, et al. Nanohydroxyapatite-reinforced chitosan composite hydrogel for bone tissue repair in vitro and in vivo. J Nanobiotechnology 2015;13:40. [Crossref] [PubMed]
- Jia S, Yang X, Song W, et al. Incorporation of osteogenic and angiogenic small interfering RNAs into chitosan sponge for bone tissue engineering. Int J Nanomedicine 2014;9:5307-16. [PubMed]
- Sun JY, Zhao X, Illeperuma WR, et al. Highly stretchable and tough hydrogels. Nature 2012;489:133-6. [Crossref] [PubMed]
- Bendtsen ST, Wei M. Synthesis and characterization of a novel injectable alginate–collagen–hydroxyapatite hydrogel for bone tissue regeneration. J Mater Chem B 2015;3:3081-90. [Crossref]
- Bae MS, Yang DH, Lee JB, et al. Photo-cured hyaluronic acid-based hydrogels containing simvastatin as a bone tissue regeneration scaffold. Biomaterials 2011;32:8161-71. [Crossref] [PubMed]
- Wang DX, He Y, Bi L, et al. Enhancing the bioactivity of Poly(lactic-co-glycolic acid) scaffold with a nano-hydroxyapatite coating for the treatment of segmental bone defect in a rabbit model. Int J Nanomedicine 2013;8:1855-65. [Crossref] [PubMed]
- Kao CT, Lin CC, Chen YW, et al. Poly(dopamine) coating of 3D printed poly(lactic acid) scaffolds for bone tissue engineering. Mater Sci Eng C Mater Biol Appl 2015;56:165-73. [Crossref] [PubMed]
- Noorjahan A, Tan X, Liu Q, et al. Study of Cyclohexane Diffusion in Athabasca Asphaltenes. Energy Fuels 2014;28:1004-11. [Crossref]
- Liu X, Ma PX. Polymeric scaffolds for bone tissue engineering. Ann Biomed Eng 2004;32:477-86. [Crossref] [PubMed]
- Baker MI, Walsh SP, Schwartz Z, et al. A review of polyvinyl alcohol and its uses in cartilage and orthopedic applications. J Biomed Mater Res B Appl Biomater 2012;100:1451-7. [Crossref] [PubMed]
- Benoit DS, Schwartz MP, Durney AR, et al. Small functional groups for controlled differentiation of hydrogel-encapsulated human mesenchymal stem cells. Nat Mater 2008;7:816-23. [Crossref] [PubMed]
- Yang XZ, Sun TM, Dou S, et al. Block copolymer of polyphosphoester and poly(L-lactic acid) modified surface for enhancing osteoblast adhesion, proliferation, and function. Biomacromolecules 2009;10:2213-20. [Crossref] [PubMed]
- van Leeuwen AC, Huddleston Slater JJ, Gielkens PF, et al. Guided bone regeneration in rat mandibular defects using resorbable poly(trimethylene carbonate) barrier membranes. Acta Biomater 2012;8:1422-9. [Crossref] [PubMed]
- Huang MN, Wang YL, Luo YF. Biodegradable and bioactive porous polyurethanes scaffolds for bone tissue engineering. J Biomed Sci Eng 2009;2:36-40. [Crossref]
- Hsieh CY, Tsai SP, Wang DM, et al. Preparation of gamma-PGA/chitosan composite tissue engineering matrices. Biomaterials 2005;26:5617-23. [Crossref] [PubMed]
- Short AR, Koralla D, Deshmukh A, et al. Hydrogels That Allow and Facilitate Bone Repair, Remodeling, and Regeneration. J Mater Chem B Mater Biol Med 2015;3:7818-7830. [Crossref] [PubMed]
- Priddy LB, Chaudhuri O, Stevens HY, et al. Oxidized alginate hydrogels for bone morphogenetic protein-2 delivery in long bone defects. Acta Biomater 2014;10:4390-9. [Crossref] [PubMed]
- Bae MS, Ohe JY, Lee JB, et al. Photo-cured hyaluronic acid-based hydrogels containing growth and differentiation factor 5 (GDF-5) for bone tissue regeneration. Bone 2014;59:189-98. [Crossref] [PubMed]
- Nonoyama T, Wada S, Kiyama R, et al. Double-Network Hydrogels Strongly Bondable to Bones by Spontaneous Osteogenesis Penetration. Adv Mater 2016;28:6740-5. [Crossref] [PubMed]
- Temenoff JS, Mikos AG. Injectable biodegradable materials for orthopedic tissue engineering. Biomaterials 2000;21:2405-12. [Crossref] [PubMed]
- Amini AA, Nair LS. Injectable hydrogels for bone and cartilage repair. Biomed Mater 2012;7:024105 [Crossref] [PubMed]
- Bonzani IC, Adhikari R, Houshyar S, et al. Synthesis of two-component injectable polyurethanes for bone tissue engineering. Biomaterials 2007;28:423-33. [Crossref] [PubMed]
- Fu S, Ni P, Wang B, et al. Injectable and thermo-sensitive PEG-PCL-PEG copolymer/collagen/n-HA hydrogel composite for guided bone regeneration. Biomaterials 2012;33:4801-9. [Crossref] [PubMed]
- Gyawali D, Nair P, Zhang Y, et al. Citric acid-derived in situ crosslinkable biodegradable polymers for cell delivery. Biomaterials 2010;31:9092-105. [Crossref] [PubMed]
- Dadsetan M, Guda T, Runge MB, et al. Effect of calcium phosphate coating and rhBMP-2 on bone regeneration in rabbit calvaria using poly(propylene fumarate) scaffolds. Acta Biomater 2015;18:9-20. [Crossref] [PubMed]
- Jiang Q, Reddy N, Zhang S, et al. Water-stable electrospun collagen fibers from a non-toxic solvent and crosslinking system. J Biomed Mater Res A 2013;101:1237-47. [Crossref] [PubMed]
- Chen DC, Lai YL, Lee SY, et al. Osteoblastic response to collagen scaffolds varied in freezing temperature and glutaraldehyde crosslinking. J Biomed Mater Res A 2007;80:399-409. [Crossref] [PubMed]
- Hennink WE, van Nostrum CF. Novel crosslinking methods to design hydrogels. Adv Drug Deliv Rev 2002;54:13-36. [Crossref] [PubMed]
- Bigi A, Cojazzi G, Panzavolta S, et al. Stabilization of gelatin films by crosslinking with genipin. Biomaterials 2002;23:4827-32. [Crossref] [PubMed]
- Norowski PA Jr, Fujiwara T, Clem WC, et al. Novel naturally crosslinked electrospun nanofibrous chitosan mats for guided bone regeneration membranes: material characterization and cytocompatibility. J Tissue Eng Regen Med 2015;9:577-83. [Crossref] [PubMed]
- Han B, Jaurequi J, Tang BW, et al. Proanthocyanidin: a natural crosslinking reagent for stabilizing collagen matrices. J Biomed Mater Res A 2003;65:118-24. [Crossref] [PubMed]
- Wu L, Ding J. Effects of porosity and pore size on in vitro degradation of three-dimensional porous poly(D,L-lactide-co-glycolide) scaffolds for tissue engineering. J Biomed Mater Res A 2005;75:767-77. [Crossref] [PubMed]
- Mitragotri S, Lahann J. Physical approaches to biomaterial design. Nat Mater 2009;8:15-23. [Crossref] [PubMed]
- McBeath R, Pirone DM, Nelson CM, et al. Cell shape, cytoskeletal tension, and RhoA regulate stem cell lineage commitment. Dev Cell 2004;6:483-95. [Crossref] [PubMed]
- Kilian KA, Bugarija B, Lahn BT, et al. Geometric cues for directing the differentiation of mesenchymal stem cells. Proc Natl Acad Sci U S A 2010;107:4872-7. [Crossref] [PubMed]
- Yoon JK, Kim HN, Bhang SH, et al. Enhanced Bone Repair by Guided Osteoblast Recruitment Using Topographically Defined Implant. Tissue Eng Part A 2016;22:654-64. [Crossref] [PubMed]
- Kachouie NN, Du Y, Bae H, et al. Directed assembly of cell-laden hydrogels for engineering functional tissues. Organogenesis 2010;6:234-44. [Crossref] [PubMed]
- Kim SS, Sun Park M, Jeon O, et al. Poly(lactide-co-glycolide)/hydroxyapatite composite scaffolds for bone tissue engineering. Biomaterials 2006;27:1399-409. [Crossref] [PubMed]
- Nie L, Chen D, Suo J, et al. Physicochemical characterization and biocompatibility in vitro of biphasic calcium phosphate/polyvinyl alcohol scaffolds prepared by freeze-drying method for bone tissue engineering applications. Colloids Surf B Biointerfaces 2012;100:169-76. [Crossref] [PubMed]
- Xia Z, Yu X, Jiang X, et al. Fabrication and characterization of biomimetic collagen-apatite scaffolds with tunable structures for bone tissue engineering. Acta Biomater 2013;9:7308-19. [Crossref] [PubMed]
- Martínez-Vázquez FJ, Cabañas MV, Paris J, et al. Fabrication of novel Si-doped hydroxyapatite/gelatine scaffolds by rapid prototyping for drug delivery and bone regeneration. Acta Biomater 2015;15:200-9. [Crossref] [PubMed]
- Rajzer I, Menaszek E, Kwiatkowski R, et al. Electrospun gelatin/poly(ε-caprolactone) fibrous scaffold modified with calcium phosphate for bone tissue engineering. Mater Sci Eng C Mater Biol Appl 2014;44:183-90. [Crossref] [PubMed]
- Ribeiro N, Sousa SR, van Blitterswijk CA, et al. A biocomposite of collagen nanofibers and nanohydroxyapatite for bone regeneration. Biofabrication 2014;6:035015 [Crossref] [PubMed]
- Lei B, Shin KH, Noh DY, et al. Nanofibrous gelatin–silica hybrid scaffolds mimicking the native extracellular matrix (ECM) using thermally induced phase separation. J Mater Chem 2012;22:14133-40. [Crossref]
- Yeung T, Georges PC, Flanagan LA, et al. Effects of substrate stiffness on cell morphology, cytoskeletal structure, and adhesion. Cell Motil Cytoskeleton 2005;60:24-34. [Crossref] [PubMed]
- Guilak F, Cohen DM, Estes BT, et al. Control of stem cell fate by physical interactions with the extracellular matrix. Cell Stem Cell 2009;5:17-26. [Crossref] [PubMed]
- Lo CM, Wang HB, Dembo M, et al. Cell movement is guided by the rigidity of the substrate. Biophys J 2000;79:144-52. [Crossref] [PubMed]
- Kong HJ, Liu J, Riddle K, et al. Non-viral gene delivery regulated by stiffness of cell adhesion substrates. Nat Mater 2005;4:460-4. [Crossref] [PubMed]
- Khatiwala CB, Peyton SR, Putnam AJ. Intrinsic mechanical properties of the extracellular matrix affect the behavior of pre-osteoblastic MC3T3-E1 cells. Am J Physiol Cell Physiol 2006;290:C1640-50. [Crossref] [PubMed]
- Engler AJ, Sen S, Sweeney HL, et al. Matrix elasticity directs stem cell lineage specification. Cell 2006;126:677-89. [Crossref] [PubMed]
- Discher DE, Janmey P, Wang YL. Tissue cells feel and respond to the stiffness of their substrate. Science 2005;310:1139-43. [Crossref] [PubMed]
- Han F, Zhu C, Guo Q, et al. Cellular modulation by the elasticity of biomaterials. J Mater Chem B 2016;4:9-26. [Crossref]
- Wang PY, Tsai WB, Voelcker NH. Screening of rat mesenchymal stem cell behaviour on polydimethylsiloxane stiffness gradients. Acta Biomater 2012;8:519-30. [Crossref] [PubMed]
- Kim TH, An DB, Oh SH, et al. Creating stiffness gradient polyvinyl alcohol hydrogel using a simple gradual freezing-thawing method to investigate stem cell differentiation behaviors. Biomaterials 2015;40:51-60. [Crossref] [PubMed]
- Ma Z, He W, Yong T, et al. Grafting of gelatin on electrospun poly(caprolactone) nanofibers to improve endothelial cell spreading and proliferation and to control cell Orientation. Tissue Eng 2005;11:1149-58. [Crossref] [PubMed]
- Lee YJ, Lee JH, Cho HJ, et al. Electrospun fibers immobilized with bone forming peptide-1 derived from BMP7 for guided bone regeneration. Biomaterials 2013;34:5059-69. [Crossref] [PubMed]
- Bosetti M, Fusaro L, Nicolì E, et al. Poly-L-lactide acid-modified scaffolds for osteoinduction and osteoconduction. J Biomed Mater Res A 2014;102:3531-9. [Crossref] [PubMed]
- Niu X, Feng Q, Wang M, et al. Porous nano-HA/collagen/PLLA scaffold containing chitosan microspheres for controlled delivery of synthetic peptide derived from BMP-2. J Control Release 2009;134:111-7. [Crossref] [PubMed]
- Seo BB, Choi H, Koh JT, et al. Sustained BMP-2 delivery and injectable bone regeneration using thermosensitive polymeric nanoparticle hydrogel bearing dual interactions with BMP-2. J Control Release 2015;209:67-76. [Crossref] [PubMed]
- Bouyer M, Guillot R, Lavaud J, et al. Surface delivery of tunable doses of BMP-2 from an adaptable polymeric scaffold induces volumetric bone regeneration. Biomaterials 2016;104:168-81. [Crossref] [PubMed]
- Shin YM, La WG, Lee MS, et al. Extracellular matrix-inspired BMP-2-delivering biodegradable fibrous particles for bone tissue engineering. J Mater Chem B 2015;3:8375-82. [Crossref]
- Matsumoto G, Hoshino J, Kinoshita Y, et al. Alveolar bone regeneration using poly-(lactic acid-co-glycolic acid-co-ε-caprolactone) porous membrane with collagen sponge containing basic fibroblast growth factor: an experimental study in the dog. J Biomater Appl 2012;27:485-93. [Crossref] [PubMed]
- Raphel J, Holodniy M, Goodman SB, et al. Multifunctional coatings to simultaneously promote osseointegration and prevent infection of orthopaedic implants. Biomaterials 2016;84:301-14. [Crossref] [PubMed]
- Kingshott P, Wei J, Bagge-Ravn D, et al. Covalent Attachment of Poly(ethylene glycol) to Surfaces, Critical for Reducing Bacterial Adhesion. Langmuir 2003;19:6912-21. [Crossref]
- Yang F, Williams CG, Wang DA, et al. The effect of incorporating RGD adhesive peptide in polyethylene glycol diacrylate hydrogel on osteogenesis of bone marrow stromal cells. Biomaterials 2005;26:5991-8. [Crossref] [PubMed]
- Schneider OD, Loher S, Brunner TJ, et al. Flexible, silver containing nanocomposites for the repair of bone defects: antimicrobial effect against E. coli infection and comparison to tetracycline containing scaffolds. J Mater Chem 2008;18:2679-84. [Crossref]
- Huang D, Zuo Y, Zou Q, et al. Antibacterial chitosan coating on nano-hydroxyapatite/polyamide66 porous bone scaffold for drug delivery. J Biomater Sci Polym Ed 2011;22:931-44. [Crossref] [PubMed]
- Tommasi G, Perni S, Prokopovich P. An Injectable Hydrogel as Bone Graft Material with Added Antimicrobial Properties. Tissue Eng Part A 2016;22:862-72. [Crossref] [PubMed]
- Chen Z, Klein T, Murray RZ, et al. Osteoimmunomodulation for the development of advanced bone biomaterials. Materials Today 2016;19:304-21. [Crossref]
- Franz S, Rammelt S, Scharnweber D, et al. Immune responses to implants - a review of the implications for the design of immunomodulatory biomaterials. Biomaterials 2011;32:6692-709. [Crossref] [PubMed]
- Chen Z, Klein T, Murray RZ, et al. Osteoimmunomodulation for the development of advanced bone biomaterials. Materials Today 2016;19:304-21. [Crossref]
- Battiston KG, Ouyang B, Labow RS, et al. Monocyte/macrophage cytokine activity regulates vascular smooth muscle cell function within a degradable polyurethane scaffold. Acta Biomater 2014;10:1146-55. [Crossref] [PubMed]
- Brodbeck WG, Patel J, Voskerician G, et al. Biomaterial adherent macrophage apoptosis is increased by hydrophilic and anionic substrates in vivo. Proc Natl Acad Sci U S A 2002;99:10287-92. [Crossref] [PubMed]
- McWhorter FY, Wang T, Nguyen P, et al. Modulation of macrophage phenotype by cell shape. Proc Natl Acad Sci U S A 2013;110:17253-8. [Crossref] [PubMed]
- Garg K, Pullen NA, Oskeritzian CA, et al. Macrophage functional polarization (M1/M2) in response to varying fiber and pore dimensions of electrospun scaffolds. Biomaterials 2013;34:4439-51. [Crossref] [PubMed]
- Blakney AK, Swartzlander MD, Bryant SJ. The effects of substrate stiffness on the in vitro activation of macrophages and in vivo host response to poly(ethylene glycol)-based hydrogels. J Biomed Mater Res A 2012;100:1375-86. [Crossref] [PubMed]
- Patil SD, Papadimitrakopoulos F, Burgess DJ. Dexamethasone-loaded poly(lactic-co-glycolic) acid microspheres/poly(vinyl alcohol) hydrogel composite coatings for inflammation control. Diabetes Technol Ther 2004;6:887-97. [Crossref] [PubMed]
- Yamaguchi M, Weitzmann MN. The intact strontium ranelate complex stimulates osteoblastogenesis and suppresses osteoclastogenesis by antagonizing NF-κB activation. Mol Cell Biochem 2012;359:399-407. [Crossref] [PubMed]
Cite this article as: Shi C, Yuan Z, Han F, Zhu C, Li B. Polymeric biomaterials for bone regeneration. Ann Joint 2016;1:27.